How do you predict an earthquake?
Expert reviewers
Professor Suzanne O'Reilly AM FAA
Director, ARC Centre of Excellence for Core to Crust Fluid Systems and Earth and Planetary Sciences
Macquarie University
Essentials
- Earthquakes are the result of the release of stress/strain that builds up as Earth’s tectonic plates move and grind against each other.
- Although some regions around the world are definitely more prone to earthquakes than others, it is not possible to accurately predict exactly where or when earthquakes will occur.
- Research is ongoing to monitor and map the accumulation of strain within Earth’s crust to improve understandings of the processes that cause earthquakes.
Terra firma: from the Latin for ‘firm land’. But that firm land upon which we live, and build our houses and cities, is sometimes not so firma. When a big earthquake hits, it can be devastating—entire cities can be levelled, not to mention the loss of human lives. Obviously we’d like to prevent this sort of destruction, so why don’t we predict these events better so we can prepare for them?
Short answer is: we can’t. While we can definitely identify areas of the world where earthquakes are more likely to occur, it’s actually not currently possible to predict exactly when or where an earthquake is going to happen.
To understand why, we need to know exactly what an earthquake is, and what causes them. Our understanding of the inner workings of our planet is constantly improving, so let’s have a look at what we do know about earthquakes, and the techniques used to try and mitigate the devastation they can cause.
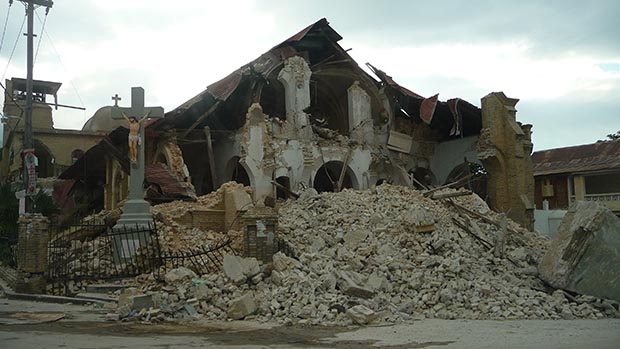
What causes an earthqake?
An earthquake is the movement of Earth’s crust that results from the sudden release of stress that has built up deep underground over time. So where does this stress come from? To answer this, we need to understand a little about Earth’s structure.
The structure of our planet
At the centre of our planet—around 4,000 kilometres beneath the surface—is an extremely hot, solid core, made mostly of iron and maybe a little bit of nickel. At least, we’re pretty sure that’s what it’s made of, but no one’s actually been there to check! Surrounding the inner core, is a molten outer core, also thought to be made of mostly iron and some nickel. Around this is another high-temperature layer, called the convecting mantle. This layer isn’t exactly molten, but it’s plastic. This enables convection currents, driven by heat from the planet’s core, to ‘flow’ slowly within it.
The uppermost section of the mantle is cold and relatively brittle, and above this layer is the crust, the outermost layer of the planet. This coupled layer of crust and uppermost mantle is called the lithosphere. Although it feels solid as we walk around on it, the lithosphere is actually very thin compared to the other layers of Earth—it ranges from less than 10 kilometres to more than 200 kilometres thick in different areas.
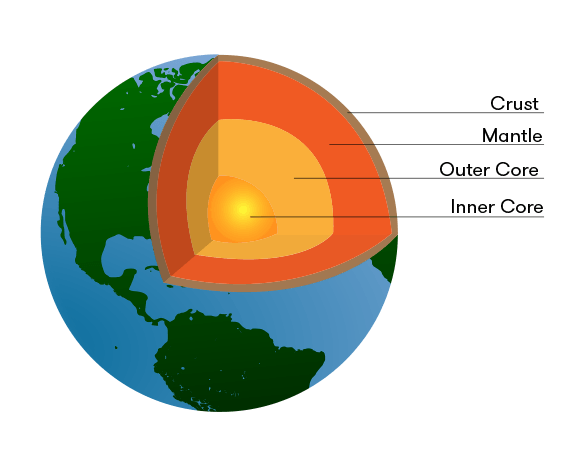
Earth’s crust is not a single, unbroken layer surrounding Earth like a smooth eggshell. It’s made of sections, called tectonic plates. The word tectonic comes from the Greek word τεκτονικός, which means ‘pertaining to building’. The tectonic plates are made up of the crust and the cold and brittle uppermost part of the mantle.
As these plates are situated on top of the slowly flowing hot mantle, they don’t stay still—over time they migrate around the planet, sometimes grinding against each other, or squishing into each other to build mountain ranges. In other places where plates are moving towards each other, one plate is forced underneath the other plate. These are known as subduction zones, and the world’s largest earthquakes occur in these regions.
In areas of the Pacific and Indian oceans, the tectonic plates are moving away from each other, and new fresh material melted from the mantle oozes out from between the plates in the middle of these oceans as lavas to form brand new oceanic crust.
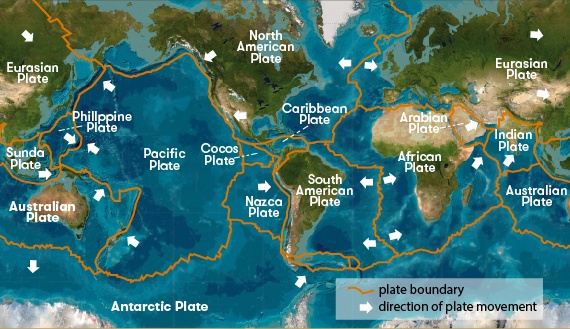
So with all this dynamic movement constantly taking place all over the planet, big plates of rock moving around and crashing into and against each other, it’s no wonder that sometimes it gets a little … unstable. As the plates move relative to each other, huge amounts of stress can build up over long periods of time. Eventually there comes a point when all the accumulated stress is suddenly released: rocks break, huge sections of crust are cracked and displaced—our solid ground becomes not so solid. These movements result in waves of energy, called seismic waves, radiating out in all directions. They travel into the interior of the planet and also towards Earth’s surface.
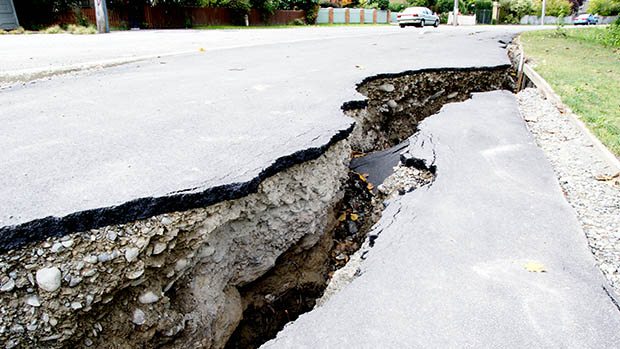
It’s also no surprise that the most seismically active regions of the planet are found along the boundaries of the tectonic plates. But not all earthquakes occur along the tectonic plate boundaries. The entire continent of Australia is in the middle of a tectonic plate, with no part of it near a major plate boundary.
Australia should therefore be reasonably safe from big earthquakes, but they still occur from time to time. In 1989 an earthquake registering magnitude 5.4 killed 13 people in Newcastle, most of them in a single building that collapsed. According to data on the Geoscience Australia Earthquake Catalogue, during the past 50 years (up to 11 November 2016) around 144 earthquakes of magnitudes greater than 5 have been recorded in Australia, but only 8 with magnitude greater than 6 and none with magnitude greater than 7. Geoscience Australia also records the magnitudes and locations of recent earthquakes within the broader Australian region.
Looking back through the rock record, geologists have identified an earthquake event that occurred on the Cadell Fault, in southern New South Wales. This earthquake probably had a magnitude around 7 or greater and the ground it displaced formed a steep scarp, having the effect of damming the Murray River, which lies on the fault. This formed a large lake until the river began to flow again to the south around the scarp.
Understanding earthquake hazard
The cause of earthquakes is the accumulation of stress that builds up within Earth’s lithosphere: in a region that’s virtually impossible for us to see, and over time scales that can be tricky for us to monitor. This makes it hard to figure out exactly where or when an earthquake might occur. There are a few methods used for earthquake forecasting though—and note the use of the specific term ‘forecasting’. It’s never called earthquake ‘prediction’ as this word implies a level of accuracy that is simply not yet achievable.
The best method currently available to scientists and planners regarding earthquake forecasting is the record of seismic events that have occurred in an area in the past. Looking at the frequency of events of particular magnitude over time, scientists can calculate the statistical probability of similar events occurring within a certain timeframe in the future.
Along with these statistical calculations, a lot of work has been done examining the geological record of earthquake-prone regions of the crust to understand the how many earthquake events have occurred, when they occurred and what size they were. Looking at the rock record in this way provides scientists with a much longer-term record to complement historical observations.
Using these insights from the geological record, scientists can then make assessments of modern day environments and how their geological setting will influence the likelihood and potential impacts of earthquakes that may occur there.
Models of potential future events and their impacts are also constructed, to create possible scenarios of how an earthquake hazard may pan out. These are used to inform emergency planning decisions—ensuring that the community is aware of potential hazards and equipped with an appropriate response plan is an essential part of earthquake preparedness.
This modelling work projecting earthquake scenarios is combined with extensive surveys of the damage caused by past events and assessments of how buildings responded to inform the development of building standards. A comprehensive building code has been in place in Australia since 1995 but much of our infrastructure pre-dates this, and so efforts are also concentrated on developing ways to retro-fit existing buildings.
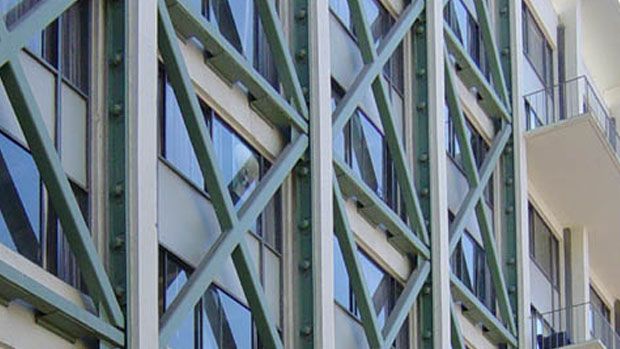
But if we know that earthquakes are caused by the release of stress that builds up when tectonic plates move against each other, then why can’t we just monitor the plates’ movements and figure out how much stress is building up and when it’s going to reach that critical release point?
Well, we’re trying.
Monitoring Earth's crust
As part of the AuScope program, more than 100 continuously operating satellite sensors have been put in place all over mainland Australia, as well as on several of Australia’s island territories. The sensors track the global navigation satellite systems (GNSS), including the United States GPS satellites, the Russian GLONAS system, the Chinese Beidou system, Japan’s QZSS and the European Union’s Galileo system. This tracking data enables scientists to determine highly accurate locations for the senors, and how these locations change over time (as the tectonic plates move). The data also supports the International GNSS Service and a wide range of other applications.
The sensors themselves are unassuming—a simple-looking device atop a short concrete post that is attached directly to the bedrock. Yet despite their less-than-impressive appearance, the data they are collectively amassing is nothing short of stupendous. The continual stream of data from the sensors means scientists can track the movements of the Australian continental plate with millimetre accuracy, watching it in real-time as it drifts inexorably northward at a rate of around 7 centimetres per year.
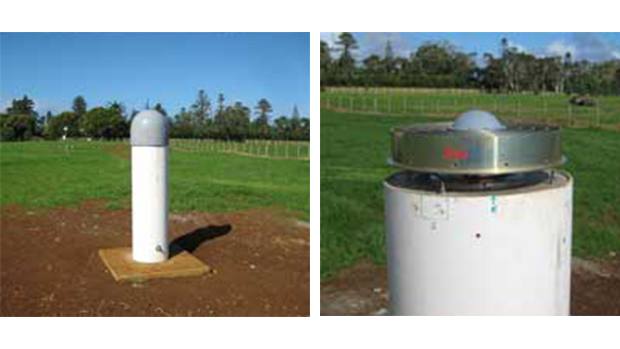
But how does this relate to earthquakes? Well, by comparing the rate of movement of each individual sensor compared to the other sensors, scientists can see which areas of the plate are moving faster than others. By picking up ‘distortions’ in the plate’s movements, they can use this to figure out where stress and strain might be accumulating—stress is the force being exerted on the rocks, and strain is the deformation of the rocks in response to the stress. Monitoring these factors can provide clues as to where an earthquake might be likely to occur. And although they don’t know yet what the critical point for strain accumulation might be—how much strain the crust can accommodate before an earthquake happens—this sort of baseline data is essential to putting together the entire picture of how our planet works.
This technique has worked well monitoring active plate boundary areas, but as our plate is moving (and deforming) so slowly, it will take quite a while before we can gain significant insights into our crust. The system has been up and running for around eight years, yet it will take decades for patterns in the data to emerge that allow improved forecasting.
Understanding aftershocks
Earthquakes generally don’t like solitude—they occur in clusters. The main event can be presaged by smaller ‘foreshocks’ but there’s no real way of ‘reading’ the preliminary tremors to figure out when the big one might be coming.
Following the main event is usually a series of after-shocks, smaller tremors that don’t pack the punch of the main earthquake but they can still cause lots of damage, especially as they impact upon buildings and infrastructure that has been destabilised by the initial earthquake.
Generally, the bigger the earthquake, the more aftershocks will follow it, and the greater they will be. Just as a magnitude 7 earthquake is 10 times more powerful than one that measures 6, it will also produce 10 times as many aftershocks.
Aftershocks generally decrease in frequency over time, and there have been several attempts to define how aftershocks pan out after the main quake. Again, our understanding of the geological processes that cause the aftershocks is not yet detailed enough to accurately predict the size and frequency of aftershocks, but statistical models have been developed using data recorded from past earthquakes that are pretty good at forecasting aftershocks.
These are based on Omori’s Law, developed in 1894 by a Japanese seismologist called Fusakichi Omori who noted that the frequency of aftershocks generally decreases by the reciprocal of time (in days) following the main quake. This means that if there are 50 aftershocks during the first day, there will likely be around 25 (1/2 x 50) on day two, 16 (1/3 x 50) on day 3, 12 (1/4 x 50) on day four and so on.
The other complicating factor is that seismic waves can travel very differently through different types of rock—so the way an earthquake propagates through granite bedrock is very different to its impact on sandstone or limestone. This is particularly the case for the shallowest (10–100 metres) of the crust, where soil and soft sediments can slow down the seismic waves. This causes them to bunch up, amplifying the impact they ultimately have.
Saturated soils—these are soils where all the pore spaces between soil grains are filled with water—that occur below the water table can also become ‘liquefied’ during an earthquake. The pressure exerted by the seismic waves causes the soil to lose its structural integrity, and it flows like a liquid. This has obvious implications for any buildings that happen to be built upon it. Interestingly, as liquids do not propagate the energy of seismic waves, the liquefied soil acts to dampen further impacts of the earthquake.
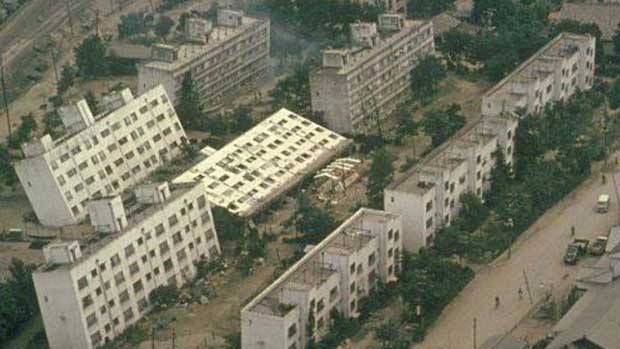
There’s still a lot to learn when it comes to understanding how our dynamic planet works and how its inner workings affect us on its surface. But we’re working on it, and hopefully one day not too far into the future we’ll have a rock-solid idea about what’s going on deep underneath our feet.
Geoscience Australia licensing details:
© Commonwealth of Australia (Geoscience Australia) 2016. This product is released under the Creative Commons Attribution 4.0 International Licence.