One more piece in the puzzle of the universe—a Higgs-shaped one
Expert reviewers
Director, ARC Centre of Excellence for Physics at the Terascale, School of Physics
The University of Melbourne
Professor Bruce McKellar AC FAA
Honorary Professorial Fellow, School of Physics
The University of Melbourne
People have always been curious about how the world works, and what everything is fundamentally made of. The ancient Greeks thought it all boiled down to four elemental substances: earth, air, fire and water. Over the centuries, physicists have taken these concepts and run amok. Modern day particle physics is concerned with how the world works on a sub-atomic (really really small) scale.
For a while, we thought that the atom GLOSSARY atomThe fundamental unit of all matter consisting of a nucleus of protons and neutrons surrounded by orbiting electrons (or in the case of hydrogen, just one electron). was the smallest component of matter, and could not be broken up into anything smaller (the name comes from the Greek word atomon, which means ‘that which cannot be divided’) and that a hydrogen atom was the smallest possible particle of matter. We now know that the fundamental world extends far beyond the basics of earth, air, fire and water, and there are a lot of things smaller than a hydrogen atom. In fact, there are more than 200 types of sub-atomic particles, which interact in very complex ways.
The Standard Model
Explaining how the world works
Of the 200 sub-atomic particles that we know about, there are some that cannot be split into smaller components, and these are called elementary particles. These elementary particles combine to form composite particles. There are also four fundamental forces, or fields, within the universe: the strong nuclear force, the weak nuclear force, the electromagnetic force and the gravitational force. These forces act upon different types of particles and control how they interact with each other.
The Standard Model is theoretical physicists’ best attempt yet to describe the elementary particles, and to explain how three of the four forces govern the way they behave. The model is a sort of instruction manual for the universe, with 12 elementary particles of matter, and the fundamental forces provide the methods for putting the matter together.
The particles
There are two main categories of particles that make up the Standard Model: fermions and bosons. Fermions make up matter, and bosons are responsible for transmitting the forces that control how matter behaves.
Fermions are divided into quarks GLOSSARY quarksA type of fermion. Quarks are elementary particles that combine to make up the components of the nucleus of an atom (protons and neutrons). There are six different types of quark – up, down, top, bottom, charm and strange. and leptons. Quarks can be further split up into six different types: up, down, charm, strange, top, bottom. Leptons also come in six varieties: electron GLOSSARY electronA sub-atomic particle that carries a negative charge. Electrons orbit around a nucleus of protons and neutrons to make an atom. , electron neutrino, muon, muon neutrino, tau and tau neutrino. Electrons, muons and taus all have an electrical charge, which is important as it determines what forces can control them. Quarks can combine to make composite particles, such as a hadron GLOSSARY hadronA composite particle made from quarks bound together by the strong nuclear force. (remember this one for later).
Fermions
Fermions are the matter particles, the basic building blocks of everything in the universe. They are split into two groups: quarks and leptons.
Quarks
- u Up
- c Charm
- t Top
- d Down
- s Strange
- b Bottom
Leptons
- e Electron
- μ Muon
- τ Tau
- νe Electron neutrino
- νμ Muon neutrino
- ντ Tau neutrino
All matter as we know it is made from various combinations of elementary particles, stuck together by one of the fundamental forces.
Stuck together how?
This is where the bosons come in. The four fundamental forces—the weak nuclear force, the strong nuclear force, the electromagnetic force and the gravitational force each have their own specific type of boson GLOSSARY bosonA type of sub-atomic particle that mediates the forces that govern how matter particles interact. Gluons mediate the strong nuclear force; W and Z bosons mediate the weak nuclear force; photons mediate the electromagnetic force and Higgs bosons mediate interactions within the Higgs field. , known as a gauge boson. Interaction of gauge bosons results in the manifestation of the forces that control our natural world.
The weak nuclear force is controlled by the W bosons GLOSSARY W bosonsA type of boson that regulates the weak nuclear force. and Z bosons GLOSSARY Z bosonsA type of boson that regulates the weak nuclear force. and is responsible for the radioactive decay of sub-atomic particles.
The strong nuclear force is mediated by elementary bosons called gluons. It’s the strong nuclear force, and these gluon GLOSSARY gluonA type of boson that mediates the strong nuclear force. bosons, that bind quarks into composite particles like neutrons, protons, and mesons, all types of hadron.
The electromagnetic force is controlled by elementary bosons called photons GLOSSARY photonsA type of boson that mediates the electromagnetic force. . This force can act over very long ranges and governs the way electrically charged particles behave—two particles with the same charge will repel each other, while opposite charges are attracted to each other. The electromagnetic force binds protons and electrons together to make atoms and binds atoms into molecules. It causes electricity, magnetism and light, and it’s what makes solid things solid and enables us to pick them up.
As for the gravitational force, well, physicists can’t really explain exactly how this force fits into the picture … yet. There is a hypothetical boson called the graviton that controls the force of gravity, but it hasn’t yet been found. In the world of particle physics, though, the force of gravity is so weak that it does not play a role in influencing the particles. Reconciling the physics of the very tiny, sub-atomic particles and their governing forces with the physics of the macro world that we live in, where gravity is obviously very important, is a job that will probably keep physicists occupied for many rainy days to come.
Bosons
When you think bosons, think forces. Gauge bosons are the particles responsible for transmitting the forces that control how the matter particles interact. The Higgs boson is a slightly different beastie—it interacts with elementary matter particles to give them their mass.
Gauge bosons
- γ Photon
- g Gluon
- Z Z boson
- W W boson
- H Higgs
Gauge symmetries and the issue of mass
The strong and weak nuclear forces and the electromagnetic force all operate in what's known as a field. This field provides a 'connection' for the force, enabling it to do its work throughout the universe.The Standard Model requires that the weak nuclear force, the strong nuclear force, and the electromagnetic force are regulated by something called local gauge symmetries, also called local symmetries.
Being governed by these symmetries means that part of the field can be transformed independently to another part, and the overall affects and laws of that field's force will still hold true. However, maintaining this state of symmetry requires energy, and nature generally prefers to move to states of the lowest possible energy. This low energy configuration no longer exhibits this symmetry—the symmetry is ‘broken’.
In 1964, three separate papers, all discussing the concept of ‘broken symmetries’ were published in the journal Physical Review Letters—one by Peter Higgs, another by Robert Brout and François Englert and the third by Gerald Guralnik, Carl Hagen and Tom Kibble. The fact that particles can acquire mass when symmetries are broken was also mentioned in these papers, but was not their focus. In fact, it was an afterthought that Peter Higgs added to his paper only after the initial paper was rejected by the journal. The authors didn’t know it at the time, but they were laying the foundations for the concept of the Higgs mechanism, the Higgs field, and the Higgs boson. So, as with many other great scientific discoveries, the origins of the concept of the Higgs mechanism were basically accidental.
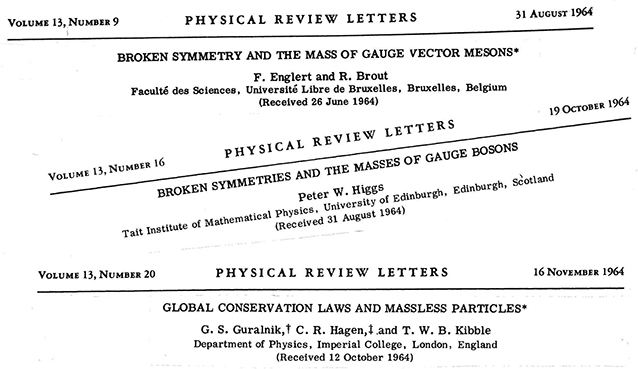
The problem of mass
Later, in 1967, the concept of the ‘electroweak’ force was developed independently by physicists Steven Weinberg and Abdus Salam. This theory combined the interactions of the weak nuclear and the electromagnetic force. The problem with this theory was that for it to work mathematically, the particles involved had to have a mass of zero, which they knew could not be true. In fact, the W and Z bosons of the weak nuclear force have large masses compared to other particles. Furthermore, if for example, electrons, had no mass, then they could not bind to a proton GLOSSARY protonA type of hadron, made from two up quarks + one down quark. Protons combine with neutrons to make up the nucleus of an atom. to make atoms, and without atoms … well … we wouldn’t be here.
The earlier work of Higgs and others describing broken symmetries and the mechanism that gave some particles mass was revisited. It turned out that as other particles of matter, such as electrons, move through the Higgs field, they interact with the Higgs bosons, which cling to or cluster around the matter particles, and give them their mass. The more Higgs boson particles that interact with the other particle, the more mass it attains.
A common analogy used to describe how the Higgs boson works is to imagine a party where all the guests are standing around, evenly distributed in the room, chatting amongst themselves. A superstar enters the room, and the guests flock to be close to the celebrity, clustering around her. As she moves through the room, more guests cluster to her, while others drop away. The people crowding around her give her momentum as she moves through the room. The superstar is an elementary particle GLOSSARY elementary particleA sub-atomic particle that can not be further divided into smaller particles. , and the guests are the Higgs bosons, giving the particle mass as they crowd around her.
Is the Higgs boson the reason I weigh 60 (or 55, or 80) kg?
While the Higgs field is responsible for giving elementary particles of matter their mass, it is not really the reason behind the number that shows on the scales when you step on them. This number is how much you weigh, which depends upon your actual mass, but also the influence of gravity (we do not exist on a sub-atomic scale, so the influence of gravity is very important in our everyday lives). If you went to the moon, you would weigh less, but your mass would still be the same.
So where do we get our mass from? We, and everything around us, are made up of atoms, which are made up of electrons, neutrons and protons. Electrons have a very small mass, and they only make a tiny contribution to the overall mass of an object. So it’s the neutrons and protons that are the most important, and they, in turn, are made up of quarks and gluon bosons. While the quarks themselves get their mass from the Higgs mechanism, this accounts for only 1 per cent of a proton’s mass. The rest of the mass comes from the energy involved in binding the quarks together. Einstein’s famous equation, E = mc2 (the amount of energy an object has (E) = its mass (m) x the speed of light squared (c2)) describes this. If you rearrange the equation, you can see how energy can translate into mass: m = E/c2.
So, the Higgs mechanism doesn’t directly dictate how much mass you, or a tree, or a rock has. But it does give quarks and electrons their mass, and without that, these particles wouldn’t be able to construct atoms, molecules and us. The Higgs mechanism is a very convenient and neat theory that has helped consolidate the Standard Model of physics, the fundamental explanation of how our world works. But physicists like to have proof for even the most elegant of theories. To really believe in these party guests, the Higgs bosons, they needed to see one of them in the flesh, so to speak.
The search for the Higgs boson
Enter the Large Hadron Collider
To find things that are really, really tiny, really, really, really big pieces of equipment are needed. The Large Hadron Collider (LHC) built by the European Organisation for Nuclear Research, or Conseil Européen pour la Recherche Nucléaire (CERN), certainly fits the bill.
This particle accelerator was built to create Higgs bosons, and also investigate other aspects of particle physics. It does this, to put it simply, by zooming particles around a ring-shaped tunnel, smashing them together and watching what happens.
The LHC is located 100 metres below ground at Geneva, Switzerland, and its 27-km-long ring spans the border of Switzerland and France. It also contains four massive detectors and an external computer grid that supports the entire network, and connects all the scientists worldwide that are working together on the Higgs hunt.
The 27 km ring—the Large part of the LHC—contains two beam paths, lined with superconducting electromagnets. These are made from coils of electrical cables chilled to around -271 °C (colder than outer space).
To make the Hadron (remember hadrons? Composite particles made from quarks stuck together by gluons of the strong nuclear force) part of the LHC, scientists take a hydrogen molecule (two hydrogen atoms stuck together) and strip off the electrons, making two protons, which are a type of hadron. Beams of protons are transmitted through each beam path around the ring, controlled by the magnets.
The beams are made of ‘bunches’ of protons, which contain around 100 billion particles each. There are nearly 3000 bunches in a beam. That makes for a lot of protons zooming around the ring, and the LHC needs to be refilled twice a day. But don’t worry, it’s not likely that the canister of hydrogen gas that supplies the protons will run out anytime soon—it only takes one cubic centimetre of hydrogen gas to refill the LHC 100,000 times.
The two beams travel in opposite directions, and as they travel around the collider ring, they go through an area of superconducting radio frequencies. These give the beams extra boosts of energy as they travel around and around the ring. At certain points, the magnets direct the beams so that they share the same beam path, making them smash into each other—this is the Collider part of the LHC. The beams are around 2 millimetres wide, and at this point they are squeezed so they become just 16 micrometers (around one fifth of the width of a hair) wide. Making the beams collide is like firing needles at each other from 10 km away and making them meet head on.
By the time they crash into each other, the beams are travelling practically at the speed of light, with an energy of 7 tera electron volts (TeV). This amount of energy is not that great in itself—one TeV is around the amount of energy produced by a flying mosquito. The special thing about the LHC is that it manages to squish that energy into a space millions and millions of times smaller than a mosquito, then smash it headfirst into another beam with the same amount of energy.
The amount of energy involved in the collision is comparable to the energy that particles possessed just after the Big Bang occurred, when the universe was so hot that all particles had an amount of thermal energy equivalent to the amount of energy that the LHC manages to produce. There is so much energy involved that new particles are created—mostly quarks, muons, neutrinos, and hopefully, Higgs bosons.
The detectors
It’s all very well to make new particles by racing protons around a huge ring then crashing them into each other, but if all this is happening close to the speed of light, 100 metres underground, in temperatures colder than outer space, it’s a bit difficult to sit back with our popcorn and watch it happen. How then are we actually going to see the particles that result from the collisions?
The LHC is fitted with four detectors, located where the collisions occur. Two of these are used to try and ‘see’ a Higgs boson: the ATLAS and CMS detectors.
ATLAS measures 46 by 25 by 25 metres and weighs 7000 tonnes. CMS is smaller, but heavier, measuring 21 by 15 by 15 metres, and weighing 12,500 tonnes.
The detectors work by either tracking the particles as they pass through the detector, or by stopping the particles in their tracks. Sub-detectors, set up like layers within the entire structure, measure the momentum, mass, energy and charge of the particles. The first layer of the detector measures the movement of particles, focusing on the very short-lived particles. Magnets in the detectors deflect the path of the particles that move through the detectors, and the charge and momentum of a particle can be figured out by measuring how much the magnet affects the particle’s movement. Calorimeters measure how much energy particles have.
What does a Higgs boson actually look like?
Here’s the kicker: sadly, we will never actually see a Higgs boson particle in the flesh. The Higgs boson only exists for one zeptosecond (that’s one sextillionth—10-21—of a second) before it decays into other particles, and the only way to confirm the existence of the Higgs boson is to see the results of this decay. There are a number of different decay patterns that can be produced by the decay of a Higgs boson. If the Higgs boson behaves the way that the Standard Model predicts it should, then these patterns should be produced in certain proportions depending on the mass of the Higgs boson.
One of the tricky things about trying to find the Higgs boson is that physicists didn’t know for sure what mass the Higgs boson actually has. Certain masses had already been ruled out by experiments at other particle colliders (the Large Electron Positron Collider, which was the LHC’s predecessor, and the Tevatron, a now-decommissioned particle collider in the USA) and the LHC, so the most recent experiments at the LHC were looking for the Higgs boson between the masses of around 120–140 GeV.
Information overload
The LHC produces something like 600 million collisions per second, which produce around 1 petabyte (1015 bytes) of data per second. This is roughly the amount of data held by 223,000 DVDs. That’s 223,000 movies, every second. This is an impossibly huge amount of data, so although it seems a bit wasteful, most of the data ends up in the rubbish bin after a quick check to see if it looks particularly interesting. Both detectors filter the data they receive, and the ATLAS system, for example, only keeps around 200 events per second, or 100 megabytes per second. Even this amounts to around 4 petabytes of data per year.
When the LHC shut down for a well-deserved break in 2013, both ATLAS and CMS had recorded data from around 3000 trillion collisions. That’s an astronomical amount of data.
The Worldwide LHC Computing Grid supplies the computing power required to analyse all this data. This grid stretches over 140 different institutions in 35 different countries.
The hunt for the Higgs involved nearly 6000 researchers working on two separate collaborations, with teams of around 3000 physicists looking at the results from both the ATLAS and CMS detectors. Many hands make light work!
The discovery
Hold the front page! It’s a Higgs! … is it? Yes!?
The first beam of hadrons was sent around the ring in 2008, 14 years after the LHC was approved for construction. In December 2011, both the ATLAS and CMS groups tentatively announced that they had indications of a hint of something that might be a Higgs boson. In July 2012, confidence had improved enough to make simultaneous announcements at CERN in Geneva, and at an international physics conference in Melbourne that they had evidence of a ‘Higgs-like’ particle. This particle pretty much ticked the Higgs boxes, but there was still enough doubt that the announcement was couched with a very big ‘maybe’.
By March 2013, the researchers had analysed another four times more data, and were happy to say that the particle they had found was looking more and more like a Higgs boson, so much so that they were prepared to drop the ‘like’ bit from the slightly awkward ‘Higgs-like particle’. And here it is (drumroll, please…):
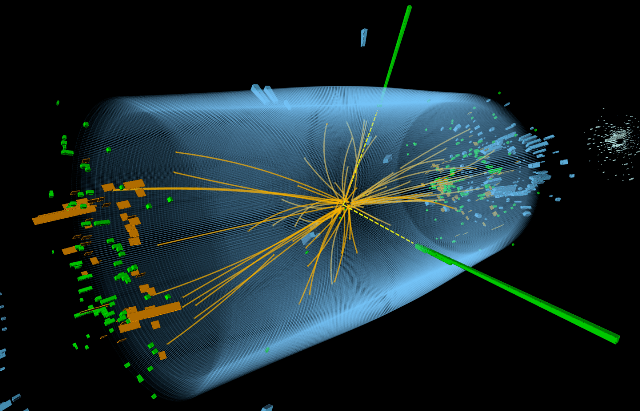
Image of an event recorded in the CMS detector showing the production of two photons (yellow dotted lines with green 'towers'). Image source: © CERN
The image shows a collision within the CMS detector that resulted in two photons, one of the possible decay patterns that could be produced by a Higgs boson. Even though there are other decay patterns that are more likely to occur, the two-photon pattern provides the clearest signal the LHC detectors are able to measure.
And after the physicists in both the ATLAS and CMS collaborations had analysed around 2500 trillion collisions, they had enough data to come up with the following plots, and got very excited.
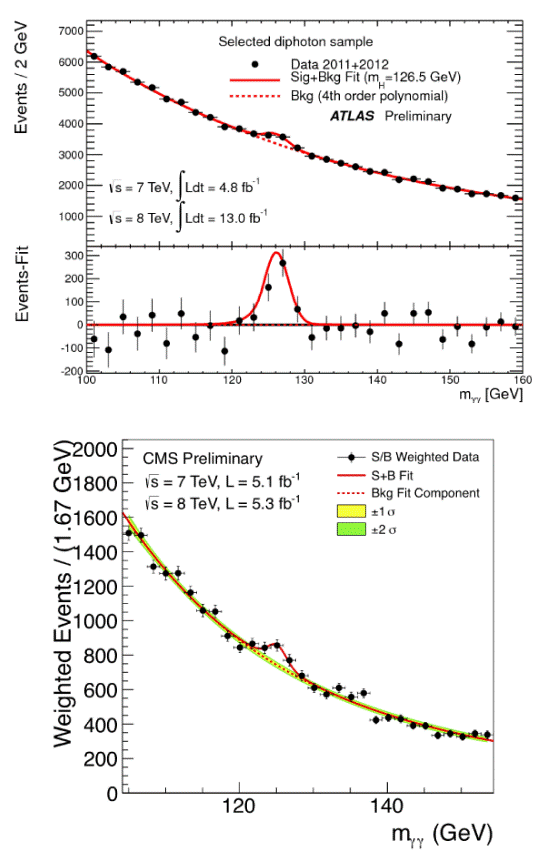
Plots showing data from the ATLAS and CMS collaborations of signals that produced a decay pattern of two photons, one of the possible decay patterns of a Higgs boson. The bumps in the curves represent signals attributed to the decay of Higgs bosons. Image source: ATLAS and CMS public data (top image; bottom image)
These plots show the data obtained by the ATLAS and CMS groups for signals that indicate a decay pattern into two photons. Don’t worry too much about the strange symbols and numbers, the main thing to notice in these plots are the bumps in the red lines that join the black dots. In the top part of the ATLAS chart and the CMS chart, the red dotted line indicates the signal that is expected to be seen from all the known processes that can cause a decay pattern of two photons. The fact that the solid red line deviates from the dotted line at the mass of 126.5 GeV (this equates to about 10−25kg) means that there is a bigger signal than can be accounted for by the known processes alone at this mass. This extra bit of signal is attributed to the decays of Higgs bosons.
The bottom part of the ATLAS chart shows this a bit more clearly—it’s a plot of the ‘residuals’. To calculate residuals, you first draw a line that best joins all the dots of your data (a line of ‘best fit’), and calculate the equation of this line. You then plug all your data back into this equation. If your line perfectly fits all your actual data, the residuals will be zero. If there are some data points that lie away from that line of best fit, then the residuals for these data points will not be zero, and the further they are away from zero, the bigger the discrepancy between the actual data and the line of best fit. In this case, the line of best fit is the line that corresponds to the signal expected by the known processes that can produce the two photon decay signal. A big bump in the residuals indicates the presence of something that strongly deviates from these known processes—extra signal, caused by the decays of Higgs bosons.
It’s very important that the results from both the ATLAS and the CMS groups show basically the same thing—the bump in the signal at 126.5 GeV. The fact that the two groups independently came up with the same result makes that result stronger; it would be very hard to believe that any bump in the signal found by the ATLAS group was actually evidence of a particle if the CMS group didn’t find the same thing.
And that's it?
Yup, that little bump in the graph is basically it. The Higgs boson. Although it might not seem terribly impressive, this is the culmination of years of work by thousands of scientists, engineers and support staff. And while it may not provide a cure for the common cold, or solve the problem of world poverty, this quest has validated some of the fundamental ideas that underpin our understanding of how the world works. This is no mean feat. Due recognition came to Peter Higgs and Francois Englert nearly 50 years after their papers discussing broken symmetries and the mass of bosons were first published, when they were awarded the 2013 Nobel Prize for Physics.
Furthermore, in the quest for answers to the big questions, science has a habit of giving us unexpected bonuses along the way. After all, CERN itself gave us the origins of the World Wide Web, back in the early 1990s. Understanding the Higgs boson, and figuring out how or if it fits the Standard Model, will undoubtedly lead to even more questions and discoveries about our universe, be they about dark matter, the notion of super symmetry or other theories that haven’t even been thought up yet. The discovery of the Higgs boson is huge, and yet it’s just one small piece in the puzzle of how our universe fits together.
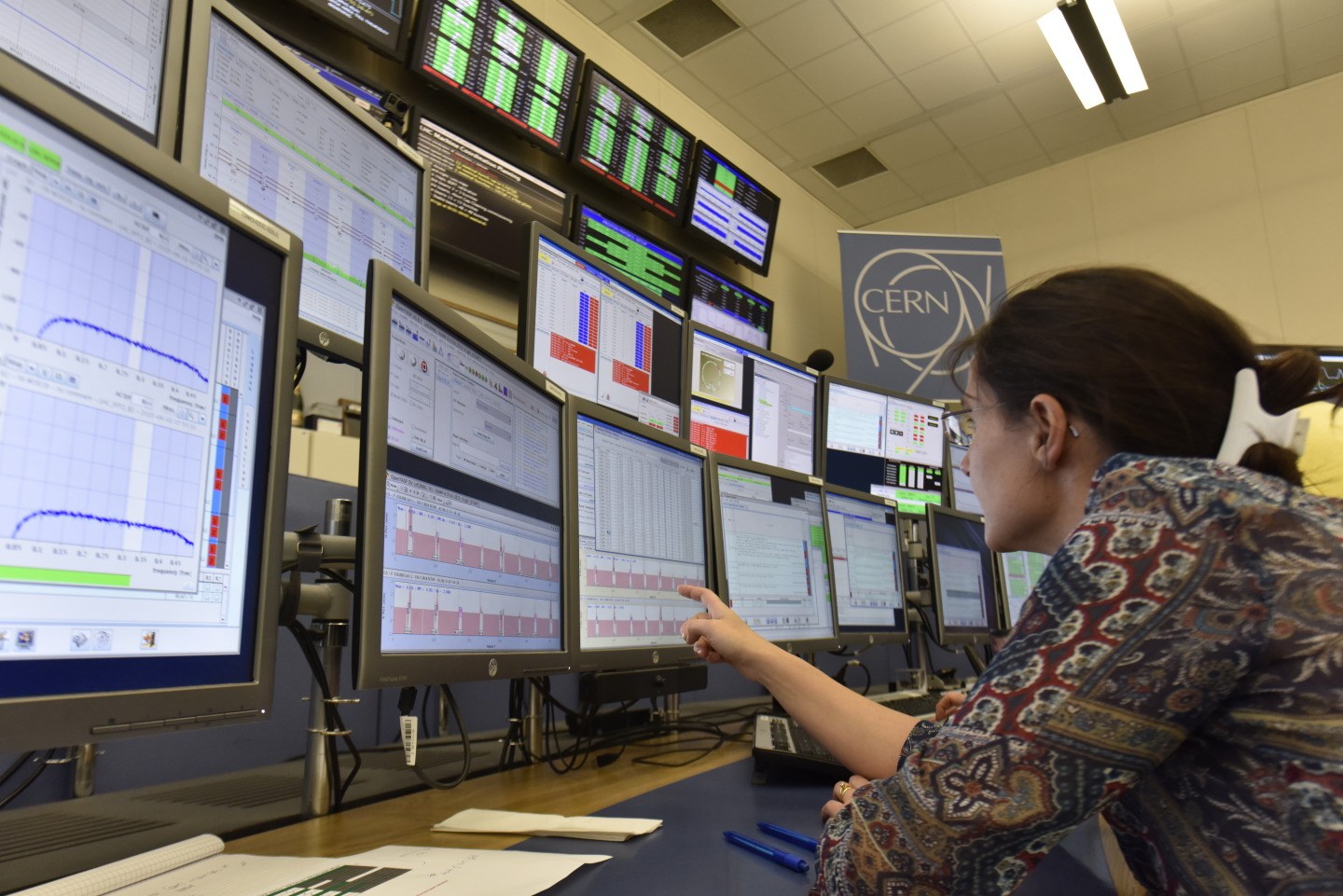
After a two-year shutdown period for planned upgrades, the second run of research began at the Large Hadron Collider in June 2015. Image source: © M.Brice / CERN.