Batteries of the future
Expert reviewers
Essentials
- New battery developments in the future will involve refinements of the current lithium-ion technology, as well as new battery chemistries.
- Battery types of the future may include lithium-air, lithium-sulphur and sodium-ion.
- Other innovations will include novel ways of charging up batteries, such as piezoelectric technologies.
- Improvements in battery technology offer a valuable storage solution for renewable energy.
Batteries have been around for hundreds of years, and they’re going to be with us for some time to come. The many and varied applications for batteries has meant numerous permutations of the electrochemical cell over the years—different metals and other materials have been used for electrodes, different substances have been used for electrolytes, and there have been different ways of putting it all together. But what does the future hold?
New battery chemistries
Variations upon a theme: new lithium-ion technologies
Silicon anode
Silicon (Si) offers a huge increase in energy storage capability when used as an anode material. When compared to the traditional graphite electrode, silicon offers a theoretical tenfold increase in capacity. However, while its lattice structure can incorporate the lithium ions needed to drive a lithium-ion battery, the inclusion of lithium ions causes a significant increase in volume—more than 300 per cent. When the battery discharges, and the lithium ions are released from the silicon anode, the silicon shrinks. Over time, this repeated expansion and shrinking fractures and cracks the silicon anode and the battery has a very short lifespan.
Solving this major problem promises to produce a battery with a significantly better energy density than a standard lithium-ion battery with a graphite anode. One option being researched is coating the silicon with graphene (single-atom-thick sheets of carbon), as the graphene sheets can ‘slide’ against each other, and compensate for the expansion and contraction of the silicon. This nearly doubles the battery’s energy density.
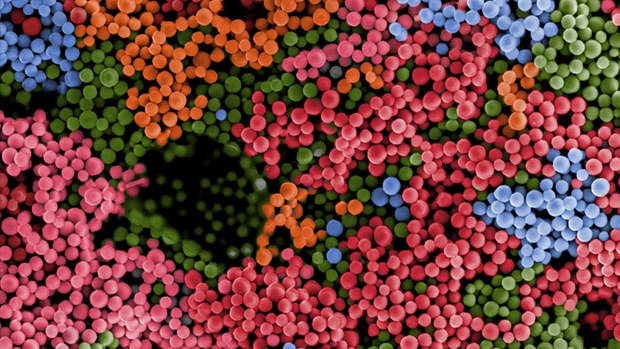
Graphene anode
Graphene could also replace graphite as a lithium-ion battery’s anode. Graphene is made of carbon atoms joined together to form a single-atom-thick sheet. While graphite is essentially formed of multiple graphene sheets stacked on top of each other, the benefits of being able to stack individual sheets of graphene allows for easier and more efficient intercalation GLOSSARY intercalationthe reversible inclusion or insertion of a molecule (or ion) into compounds with layered structures of lithium ions.
Attempting to insert lithium ions rapidly into the graphite, which is necessary for high power or fast charging applications, also causes the anode to break down. Graphene sheets could be used for high power applications, since the lithium ions do not need to tunnel through the graphite crystal to get to their insertion sites, as the sites are already open to the lithium-ion containing electrolyte. This new material has a lot of scientists worldwide trying to develop it into a new battery electrode material.
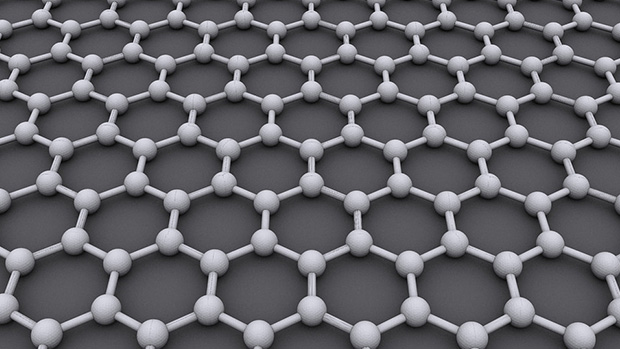
Lithium-air
What if a battery could pull its power out of thin air? A lithium-air battery would do just that, by using oxygen from the ambient atmosphere as its cathode material. This would obviously make the battery extremely light, giving it an energy density up to 10 times better than standard lithium-ion batteries—an energy density that could compete with petrol.
However, the lithium-air chemistry poses a few challenges. One is that, in its pure metal form, lithium is extremely reactive, and it is difficult to keep an anode made of lithium stable. Finding electrolyte materials that can keep the anode stable, along with preventing it from reacting with oxygen from the air, is a challenge.
There is a lot of research on electrolytes looking to solve this problem, including using polymers, ionic liquids and high salt concentration solvent mixtures. Preventing reaction with air can also be achieved by coating the electrode with a solid electrolyte like glass or ceramic. The other problem is that the reduction of oxygen results in the formation of lithium peroxide (Li2O2). This can then coat the surface of the electrodes and supresses the reaction by which lithium ions and electrons are released, quashing the battery’s power. As the cell is exposed to air from which it draws the oxygen for the cathode reaction, it’s also exposed to water vapour and carbon dioxide, which can affect the chemistry of the cell by leading to the formation of other non-conductive and inactive materials such as Li2CO3.
In recent developments, researchers at the University of Cambridge used a highly porous graphene as the cathode and added lithium iodide (LiI) and water (H2O) to the electrolyte mix. This prevented the formation of lithium peroxide, instead taking some hydrogen from the water to make lithium hydroxide (LiOH). The iodide acts as a ‘mediator’ that facilitates this reaction. The lithium hydroxide crystals fit nicely into the pores of the graphene cathode, but they do not coat its entire surface, leaving the carbon that is necessary to drive the battery’s electricity-generating reaction exposed and available to keep reacting.
When the battery is recharged, the iodide again steps in. It is oxidised to the triiodide ion (I3-). The triiodide reacts with the lithium hydroxide crystals, producing lithium ions (Li+), oxygen (O2) and water (H2O). This completely clears out the lithium hydroxide crystals from the cathode, leaving it accessible for the oxygen reduction reaction that occurs during battery discharge. Meanwhile, the lithium ions travel through the electrolyte and are redeposited on the anode as lithium metal, ready to again give up their electrons when the battery discharges.
The lithium-air technology is still a fair way from practical commercialisation. Estimates are that it’ll take around 10–20 years for it to come to fruition. The developments described above work in a system that uses pure oxygen, so researchers also need to find a way to deal with the other stuff that air contains. Gases such as carbon dioxide and nitrogen in the air react with the lithium metal in the anode to form lithium carbonates and lithium nitrates which coat the electrode surface and prevent it from working effectively.
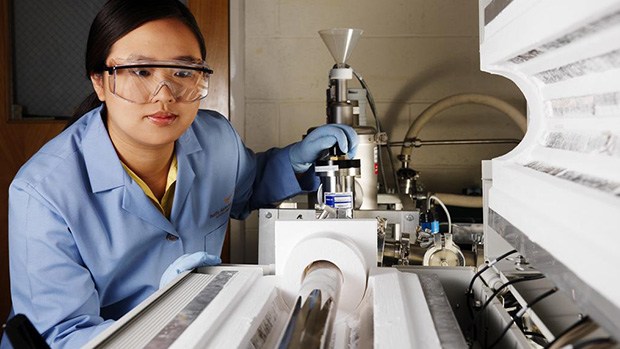
Lithium-sulphur is another promising battery chemistry for the future. Read more about it in our Nova topic on types of batteries.
Aluminium-air
As aluminium is a very common element found in Earth’s crust, a battery made from an aluminium anode and oxygen cathode promises to be very cheap. It will also be light, with a high energy density. An aluminium-air battery could potentially power an electric vehicle eight times further than the standard lithium-ion battery on a single charge.
The problem is that the chemistry that drives this battery cell results in the corrosion of the aluminium anode. As it reacts with the electrolyte, the aluminium anode undergoes an irreversible reaction to form hydrated aluminium oxide (Al(OH)3). This means the battery is not rechargeable, so either the anodes or the entire spent batteries would have to be replaced at regular intervals. The degraded anodes can be recycled to make fresh aluminium anodes.
The battery uses a water-based electrolyte, sometimes a salt solution, or a higher voltage can be obtained using potassium hydroxide (KOH).
Aluminium-air battery chemistry could potentially be improved by the development of more porous, three-dimensional structures for the aluminium anode, which would increase the surface area of aluminium that’s available to react. Another possibility is the development of aluminium alloys, where adding in just tiny amounts of other elements would help prevent the formation of the aluminium hydroxide that corrodes the anode.
Because of the difficulties in recharging aluminium-air batteries, it’s unlikely that they’ll become a real commercial prospect in the near future, however ongoing research may yet get us there in the long run. Several groups around the world are looking at alternatives, however, such as magnesium-air and zinc-air batteries. Zinc-air batteries currently power our hearing aids and cochlear ear implants, however, reversibility GLOSSARY reversibilityIt’s the reversibility of the chemical reaction in a battery that makes it rechargeable. A chemical reaction produces the electrons that are needed to make the electric current that the battery supplies to devices to make them go. That reaction has to be pushed backwards (that is, go in reverse) when the battery is recharged. is a problem.
Sodium-ion
Sodium-ion batteries work in a similar way to lithium-ion batteries, but with sodium ions instead of lithium. Advantages of sodium are that it is easy to source (just think sea water) and cheap.
While sodium ions are unlikely to compete with lithium-ion batteries to power portable devices or cars any time soon, some companies—Aquion Energy, Faradion, and Sharp Laboratories—are already producing them for use as energy storage at wind and solar farms. Smaller modules are also now being made for residential energy storage.
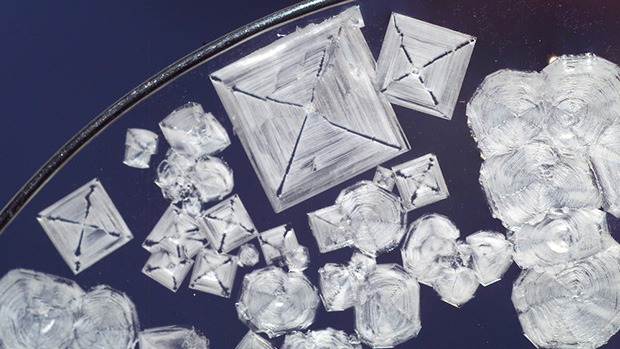
There are a few different materials that have been used for electrodes in sodium-ion batteries. Carbon is commonly used for the anode, but not in a neat arrangement of graphite as in a lithium-ion battery. When incorporated into a uniform structure, the large sodium ions get stuck and can’t get out again, making the cell reaction irreversible (and the battery unrechargeable). What’s needed to prevent this is the right pore size and distribution, and a high surface area—so ‘hard carbon’, generally made by burning sugars at high temperature, is used as the anode. The temperature is critical to getting the right properties.
A possible option is an anode made from layers of graphene interspersed with layers of phosphorene. The graphene provides elasticity and electrical conductivity to the electrode, and the phosphorene changes the structure of the electrode such that sodium can move in and out easily during recharge and discharge.
A number of different materials have been tried out as cathodes for sodium-ion batteries, but research is ongoing to find the best candidate. Recently, researchers found that a compound of sodium, iron and sulphate provided an excellent host for sodium ions. The material is formed of layers of sodium and iron interspersed with sulphate structures, which provides good spaces in which to house the sodium ions. Prussian blue GLOSSARY Prussian bluea dark-blue, crystalline, water-insoluble pigment usually used in painting and dyeing is another compound favoured for cathodes.
Entering the realm of sci-fi?
Piezoelectric
Piezoelectric materials can produce an electric charge when subjected to mechanical stress. Basically, if you squeeze, squish or press on it, that mechanical energy is converted into electrical energy.
Step-powered
Researchers have developed a small device made from a coin-sized lithium-ion battery with its separator taken out and replaced with a piezoelectric film. It can be embedded into the sole of a shoe, like your running sneaker. As you run, each time your foot hits the ground a small amount of force presses on the device, compressing the piezoelectric film in the middle. It generates a charge that causes the lithium ions to travel from the cathode to the anode—just like the recharging process that occurs when a normal lithium-ion battery is plugged into an external electricity source to recharge. The lithium ions that are pushed over to the anode react with the titanium oxide anode material to form lithium titanium oxide (LiTiO2), and are only released again when electricity is required to power a device. At this stage, it wouldn’t provide enough power to run your phone, but perhaps enough for things like GPS tracking devices. The key to this technology is developing the piezoelectric charge generation so that it can be connected to other battery types as well.
Essentially, this process involves mechanical energy being converted to chemical energy to charge the battery, then the normal chemical-to-electrical conversion when the battery is connected to an external circuit.
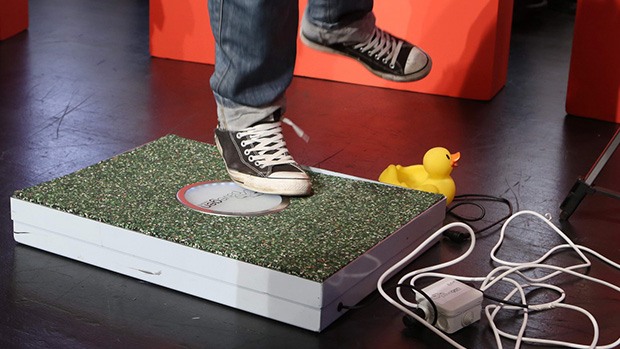
Sound-powered
Zinc oxide is a pieozoelectric material. When tiny nano-rods of it are exposed to soundwaves, they bend, creating physical stress that produces an electric current. The nano-rods are placed between metal sheets that act as the electrical contacts, drawing off the current created by the bending nano-rods. These electrical contacts can be made from common old aluminium foil.
The first attempt at building a sound-powered battery produced a device that can generate 5 volts—just by feeding off everyday noise like traffic, talking or music.
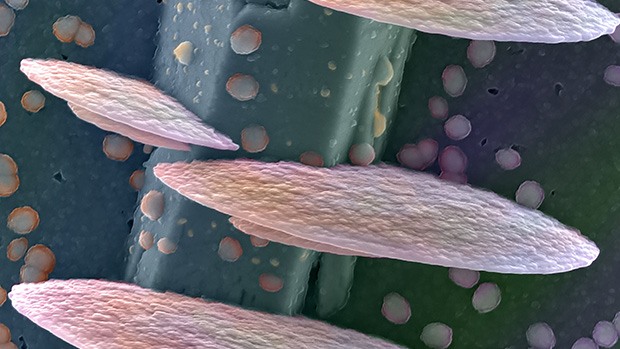
Fidgeting/friction-powered
Another option in the future might be to harness the energy produced as people go about their daily business. Researchers are working on nano-generators—devices that can harness the static electricity generated from the friction produced by two substances rubbing together.
The device would contain a sheet of metal placed alongside a sheet of terephthalate plastic, both with nano-scale structures on their surfaces. When they come into contact, an electric charge is generated, and then the current flows when the sheets bend or flex. The nano-structures on the sheets’ surfaces increase the area available for contact.
This would be made into a small patch, possibly worn as an armband. It would soak up the energy produced as we walk around the house, pick up a book or type an email.
Urine-powered
A pee-powered battery sounds ridiculous! Apparently not.
This technology is based on microbial fuel cells, which exploit the chemistry of the cell respiration process to produce electricity. After all, the chemistry underlying cell respiration is another set of redox reactions, just like those required to drive an electrochemical cell.
These particular microbial fuel cells contain live microbes that eat urine and break it down. Part of this process involves the production of electrons, which are, of course, what you need to generate an electric current. The idea is to take urine and feed it into pee-eating, microbe-containing cells, and harness the electrons produced to power electronic devices.
By placing several microbial fuel cells together in a series, researchers at the Bristol Robotics Lab have produced a device that is capable of powering a mobile phone, and hope to scale it up to a size that would be capable of providing around 12 kilowatt hours power a day.
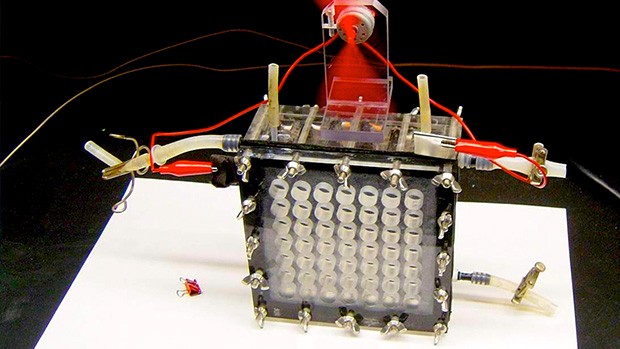
What will these batteries do for us?
Developments in technology, particularly electronics, have changed the way we live our lives. What changes are in store for our children and future generations?
Renewable power
Adequate storage of the energy produced by renewable sources such as solar and wind is seen as the ‘missing link’ that’s required to power a future without dependence on fossil fuels.
And we’re getting there. Redox flow batteries are already being used on mine sites and in remote and rural areas to store solar-produced electricity. Their bulkiness is offset by the fact that they’re cheap and reliable. Trials in Australia have shown that these batteries can be used for residential applications, such as in the smart city grids scheme, where 30,000 homes were connected to renewable energy coupled with flow batteries.
As large lithium-ion batteries become more affordable, predictions are that more and more households will be equipped with a domestic battery, something in the scale of around 6–10 kWh capacity. These batteries could take in electricity from the grid during off-peak times when the cost is cheaper, and store it for use at peak times, thus reducing home owners’ power bills.
The other option is that, for houses with solar panels installed, the batteries would store any electricity that was generated during the day, but not used immediately, for use at night-time. Combined with smart management software, a household using a battery could also feed its stored electricity into the grid at peak demand times—and be paid for it.
Domestic batteries promise to be game changers, not only for the way households use and produce electricity, but for how the national electricity grid will function in the future. We’re not quite there yet, though. Although the Tesla Powerwall battery has arrived on the scene with a huge amount of hype and fanfare, it’s certainly not the first domestic battery to be invented—various types have been around for years. The problem is that they’ve simply been too expensive for broad uptake.
The promise of the Tesla Powerwall, and the associated Tesla Gigafactory, is that it will bring costs down, which certainly ups the ante. Combined with predicted increases in grid electricity prices, we’ll almost certainly see a more widespread adoption of battery technology in our homes.
Another contender might be Oxis Energy in the United Kingdom. They’re currently testing lithium-sulphur batteries that store energy from a domestic-sized solar panel system, and hope to have them available for sale soon. Lithium-sulphur batteries are less expensive than lithium-ion batteries because sulphur is cheap and abundant. And, here in Australia, Redflow intends to soon be offering their zinc-bromide redox flow batteries as options for domestic storage as well.
Wearable batteries
Small, light and flexible, and capable of drawing power from the mechanical energy of people carrying it around? That’s the promise of wearable battery devices.
One created by CSIRO uses walking to generate electricity. A generator placed into a backpack or garments converts the mechanical energy it experiences during walking or running into electricity. This electricity is then used to charge up a fabric-based flexible battery. Using conductive fabrics to make connections between all the different components makes it possible to design ‘flexible’ new wearable technologies.
While the CSIRO technology has been demonstrated in a backpack configuration, the fabric used can be cut and shaped to any design simply by chopping it up with a pair of scissors—so the variety of possible garments or accessories is extensive.
Conclusion
Who would have thought that twitchy frog legs observed over 200 years ago would ultimately lead to devices that can power our computers, smart phones, cars and, hopefully soon, our homes? Batteries have come a long, long way over the past few hundred years and, with so many scientists around the world investigating new technologies, it’s likely they’ll take us a long, long way into the future.